Calcium is arguably one of the most important metal ions in biology (sodium and potassium are up there too!). Calcium is fundamental to muscle and nerve function, hormone secretion, blood clotting, and as we all know, a structural component of bones and teeth. It is an enzyme co-factor, a crosslinking agent, a signaling ion, and an allosteric modulator. Because of its importance, scientists have developed calcium sensors to better understand how this metal ion is trafficked and regulated. In this blog we will explore some of the most commonly used as well as newly developed calcium sensors.
Selecting a calcium sensor
Genetically encoded calcium indicators (GECIs) are fluorescent proteins linked to calcium binding proteins (e.g., calmodulin or troponin C). Their fluorescent readouts are used to monitor calcium concentrations and transients in living cells and organisms. Unlike chemical indicators, these DNA constructs can be delivered to intact tissues and organs noninvasively and target a defined population of cells (e.g., a neuron subtype) and specific subcellular compartments for measurements. All of the sensors discussed below fall into this GECI category.
For calcium sensors, one size doesn’t fit all, but never fear! That just means there is a great fit out there waiting for you. When selecting a sensor to use for your studies, you can start by asking ‘What is the biological phenomena I want to observe?’ This information, along with a little nuance discussed below, will help match your experiment with a sensor that is tuned to your parameters.
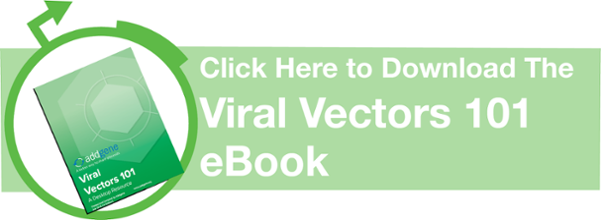
Kinetics
How fast is the process you would like to monitor? Neurons, for example, have unusually fast calcium dynamics, whereas non-neuronal systems tend to have slower, but larger, calcium changes. The GCaMP6 family of sensors are commonly used calcium reporters with different kinetic parameters. There are three GCaMP types based on their kinetics: GCaMPf (fast) GCaMPm (medium), GCaMPs (sensitive but slower). GCaMPf may be suitable for very rapid measurements, like action potentials in neurons. jGCaMP7 and jGCaMP8 are also available with improved kinetic and sensitivity parameters.
Sensitivity and Localization
The calcium concentrations in different subcellular compartments can vary greatly. Additionally, the calcium changes you will observe in your experiment could be subtle or dramatic. You want to use a calcium sensor that is most sensitive to your expected range. For example, calcium in the ER lumen reaches the sub-millimolar range, much higher than in the cytosol. Thus, for the ER you would want a low affinity calcium biosensor, so that the (1) binding of calcium, (2) allosteric rearrangements of the sensor, and (3) subsequent change in fluorescence only occurs at high calcium concentrations. This will reduce noise and ensure you are measuring the events-of-interest. Sensors suitable for ER measurements include: CEPIA (additional versions are available to simultaneously measure calcium changes in the ER, cytosol and mitochondria), the Cameleon-based FRET sensor D1ER, and GCaMPER.
Bonus: There are also Cameleon-based FRET sensors available that will localize at the plasma membrane. GCaMP-X is a nucleus-targeted GCaMP6 with an extra apoCaM-binding motif to prevent aberrant binding of the biosensor to endogenous calmodulin.
Multiplexing
Are you combining your calcium reading with other measurements, specifically fluorescent ones? Many of the available biosensors use a single GFP or CFP/YFP-derived FRET pair. If you need to free up crowded optical space for multiplexing, GECO sensors are available in green, blue, red, and far-red. There is also a ratiometric GECO sensor (GEX), where one wavelength changes with Ca while another wavelength is insensitive. Additional red sensors based on mRuby (jRCaMP1) and mApple (jRGECO1a) are also available.
Detection methods
Many calcium reporters use a single fluorescent protein (FP), requiring only a simple measurement. However, single wavelength measurements are more susceptible to artifacts and pH interference, and comparison between experiments can be more difficult due to variance in sensor expression if you aren’t working with a stably integrated biosensor. Ratiometric measurements with certain single FPs (such as GEX or blue/green CEPIA) or through FRET sensors, can reduce these unwanted variables. Twitch is a FRET-based calcium reporter derived from troponin C. It is engineered with a reduced number of calcium binding sites per sensor (to decrease unnecessary calcium buffering and to increase sensitivity), and is a great FRET sensor option if you have the setup necessary for readout.
We’ve got a live one (calcium sensors for in vivo studies)
There are several calcium reporters specially designed for the aims and requirements of in vivo studies. A particularly useful sensor for neuroscientists is CaMPARI. This sensor switches from green fluorescence to a red fluorescent state only by simultaneous (1) user-controlled illumination with violet light and (2) exposure to increased intracellular calcium. It has been applied to mark active populations of neurons in vivo to study circuit activity. CaMPARI is available for neuron-specific expression (hSyn promoter) and pan-mammalian cell expression (CAG promoter). An improved version named CaMPARI2 is available for neuron-specific expression in both zebrafish and mammalian systems.
The GCaMP6, jGCaMP7, and jGCaMP8 sensors are also suitable for in vivo studies. There are different versions optimized for neuron-specific, astrocyte-specific (GCaMP6 only) or mammalian cell expression, and compatible with constitutive or Cre-dependent expression. These sensors have also been re-engineered to measure changes in calcium specific to the neuronal cell body or the axon (GCaMP6 only). The genetic elements required for Drosophila studies have been added for jGCaMP7 and jGCaMP8.
Viral vectors
So, what do viral vectors have to do with all of these cool tools? Calcium sensors are often delivered using viral vectors! One of the most significant advantages of viral delivery is the potential for long-term expression and integration within the target cells, ensuring more consistent and reproducible results in calcium assays. There’s another crucial advantage: tissue-specific targeting. By employing various AAV serotypes, researchers can precisely direct the calcium sensor to specific tissues or even distinct cell subtypes for efficient expression and downstream monitoring. This targeted approach allows scientists to focus on specific cell populations of interest. Many of the calcium sensors described are also already packaged into viral vectors and ready to go.
Pro tip: Vectors with ready-to-use AAV preparations are labeled with the green virus particle on our biosensors page.
Whether you’re studying the brain, muscles, or intracellular landscape, remember: Keep Ca+2lm and Ca+2rry on!
Nasu Y, Shen Y, Kramer L, Campbell RE. Structure-and mechanism-guided design of single fluorescent protein-based biosensors. Nature chemical biology. 2021 May;17(5):509-18.
Inoue M. Genetically encoded calcium indicators to probe complex brain circuit dynamics in vivo. Neuroscience Research. 2021 Aug 1;169:2-8.
Suzuki J, Kanemaru K, Iino M. Genetically encoded fluorescent indicators for organellar calcium imaging. Biophysical journal. 2016 Sep 20;111(6):1119-31.
Rose T, Goltstein PM, Portugues R, Griesbeck O. Putting a finishing touch on GECIs. Frontiers in molecular neuroscience. 2014 Nov 18;7:88.
Tian L, Hires SA, Looger LL. Imaging neuronal activity with genetically encoded calcium indicators. Cold Spring Harbor Protocols. 2012 Jun 1;2012(6):pdb-top069609.
Palmer AE, Tsien RY. Measuring calcium signaling using genetically targetable fluorescent indicators. Nature protocols. 2006 Aug;1(3):1057-65.
Leave a Comment